Gravitational wave detection
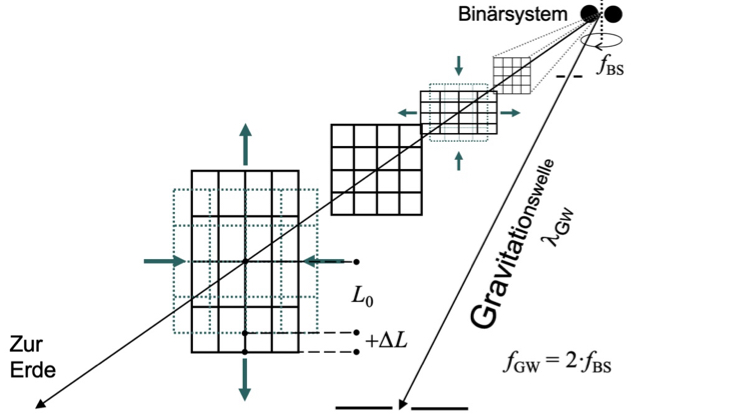
Gravitational waves are emitted by accelerated masses and manifest themselves as oscillations of space-time that propagate at the speed of light. Gravitational waves can be measured through changes in distance between "freely falling" test masses. (Meaning gravitational forces accelerate masses according to Newtons law F = m·a.) Gravitational-wave detectors are Michelson laser interferometers whose mirrors are suspended as pendulums and thus serve as test masses which behave quasi-free along the propagation direction of the laser light for small forces. The figure shows the effect of a gravitational wave on the metric of space-time.
There are several large Michelson laser interferometers worldwide built for the detection of gravitational waves. GEO600 is a German-British project with an arm length of 600m. The GEO600 was from 2012 until 2015 on the search for gravitational waves, at that time as the first GW detector with squeezed light. The technology and the squeezed laser itself were developed by Professor Schnabel and his working group from 2003 to 2010 [1,2].
The US-American LIGO project has two detectors with 4km long arms, and the Italian-French project Virgo has an arm length of 3km. The Japanese KAGRA detector also has 3km arm length. Immediately after its upgrade in 2015, Advanced LIGO observed gravitational waves for the first time ever. In the meantime, Advanced LIGO and Advanced Virgo have now reported about 100 detections. Since 2019, all events have been recorded with an improved signal-to-noise ratio using squeezed light [3,4]. Squeezed light was not part of the initial designs of neither Advanced LIGO nor Advanced Virgo, however, due to the great success of squeezed light in GEO600 [2], it was decided to be implemented as a valuable technique for reaching design sensitivity in a less challenging way.
Our group at IQP develops squeeze laser technology for gravitational-wave detectors [1-6], conducts first demonstration experiments on how squeezed and entangled light can make GW observatories even better [7,8], develops and tests machine learning ("artificial intelligence") for application in GW observatories [9].
[1] R. Schnabel, N. Mavalvala, D. E. McClelland, P. K. Lam, Quantum metrology for gravitational wave astronomy, Nature Communications 1, 121 (2010).
[2] The LIGO Scientific Collaboration (J. Abadie et al.), A gravitational wave observatory operating beyond the quantum shot-noise limit, Nature Physics 7, 962 (2011).
[3] M. Tse et al., Quantum-Enhanced Advanced LIGO Detectors in the Era of Gravitational-Wave Astronomy, Phys. Rev. Lett. 123, 231107 (2019).
[4] F. Acernese et al., Increasing the astrophysical reach of the advanced virgo detector via the application of squeezed vacuum states of light, Phys. Rev. Lett. 123, 231108 (2019).
[5] A. Schönbeck, F. Thies, R. Schnabel, 13dB Squeezed Vacuum States at 1550nm from 12mW external pump power at 775nm, Opt. Lett. 43, 110–113 (2018).
[6] C. Darsow-Fromm, J. Gurs, R. Schnabel, S. Steinlechner, Squeezed light at 2128 nm for future gravitational-wave observatories, Opt. Lett. 46, 5850 (2021).
[7] J. Südbeck, S. Steinlechner, M. Korobko, R. Schnabel, Demonstration of interferometer enhancement through EPR entanglement, Nat. Photonics 14, 240 (2020).
[8] M. Korobko, J. Südbeck, S. Steinlechner, and R. Schnabel, Mitigating Quantum Decoherence in Force Sensors by Internal Squeezing, Phys. Rev. Lett. 131, 143603 (2023).
[9] N. Heimann, J. Petermann, D. Hartwig, R. Schnabel, L. Mathey, Predicting the motion of a high-Q pendulum subject to seismic perturbations using machine learning, Appl. Phys. Lett. 122, 254101 (2023).